Experimental and Numerical Investigations on an Organic Phase Change Material Incorporated Cool Concrete Pavement
Abstract
Background:
Traditionally, cool pavements have been designed as reflective, evaporative, etc. Though the reflective pavements reduce the pavement surface temperature significantly, they increase glare, the thermal burden on pedestrian traffic, and the temperature of nearby buildings. In the case of evaporative pavements, the absence of water, reduced thermal inertia and solar reflection result in a higher pavement temperature. As a result, there has been a pressing need to investigate new low-side-effect cool pavement options.
Objective:
The study aims to analyze the effect of phase change material (PCM) incorporation on the thermal performance of concrete pavements and to develop a total enthalpy-based numerical heat transfer model for such cool pavements.
Materials and Methods:
A paraffin-based organic PCM with a melting point of 42 to 45 °C was used in this work, and expanded clay aggregate (ECA) was used as an encapsulation medium. Concrete slabs without and with the incorporation of PCM-impregnated ECAs were cast, and thermocouples were implanted in the concrete to monitor the pavement temperature continuously. A total enthalpy-based numerical heat transfer model was developed to predict the thermal performance of such cool concrete pavements.
Results:
The PCM incorporation reduced 2.24 °C in the annual average pavement surface temperature with a maximum reduction of 4.12 °C.
Conclusion:
PCM incorporation effectively reduces pavement surface temperature during the daytime and makes the pavements cooler. Increasing the encapsulating medium's porosity and the concrete slab's thermal conductivity enhances the cooling potential. However, the thermal characteristics of the encapsulating material may be neglected as their impact is less on the cooling potential.
1. INTRODUCTION
Most of the world's population depends on a sophisticated web of knowledge, institutions, and infrastructural networks to mitigate their reliance on environmental assets. The ability of these networks to adapt to changing circumstances and extreme events due to climate change will determine the future of many cities; the alternative is progressive decay or catastrophic collapse of urban areas, displacing hundreds of thousands of people and having far-reaching economic consequences. Cities are currently seen as a critical component of the global response to climate change [1, 2]. Local urbanization at various scales accounts for 37.1 to 78.3% of the warming during the past few decades [3]. Global cities are also growing fast to meet the enormous housing needs accompanying urban population expansion. As a result, the land cover, urban fabric, and urban geometry have all been dramatically altered, resulting in increased solar radiation absorption and decreased latent heat emission. Hence, the urban areas exhibit a higher air temperature than the adjacent rural/ suburban areas, called the Urban Heat Island (UHI) effect [4].
Pavement temperature significantly impacts the near-surface air temperature and covers a substantial portion of urban infrastructure [5]. Hence, minimizing the negative impacts of road pavements on the urban climate is a research priority. Further, vehicle loads and the temperature differential between the top and bottom surfaces of concrete pavement are the major causes of the development of stresses. Therefore, reducing the top pavement surface temperature will help to minimize the thermal stresses, thus extending the life of such pavements [6]. Also, reducing the pavement surface temperature in hot climatic regions provides comfort to road users. Several ways to reduce the pavement surface temperature make the pavements cooler. According to the United States Environmental Protection Agency (USEPA), “Cool pavements include a range of established and emerging technologies that communities are exploring as part of their heat island reduction efforts.” The term currently refers to paving materials that reflect more solar energy, enhance water evaporation, or have been otherwise modified to remain cooler than conventional pavements. Though traditional cool pavement solutions such as reflective pavements significantly lower pavement temperature, they raise the glare in pavements, the thermal burden on pedestrian traffic, and the temperature of neighboring buildings [7]. In the case of another traditional technology of evaporative pavements, the absence of water, lower thermal inertia and solar reflectance result in higher pavement temperatures [8], which adversely affect the thermal performance. Therefore, researchers are trying to develop new cool pavement solutions with a low environmental impact.
Phase change materials (PCMs) can store heat energy in the form of latent heat without causing a temperature rise and are currently being used in pavements to lower the temperature of the pavement surface [8]. When the temperature rises during the daytime, the PCM transforms from solid to liquid at its melting point, thereby controlling the pavement temperature from a further increase. However, during the nighttime, PCM hardens, and the stored latent heat is released. As a result, incorporating PCM into pavements lowers the temperature during the daytime and raises it during nighttime [9]. However, direct incorporation of the organic PCMs interferes with the cement hydration process and reduces the mechanical strength of concrete. Hence, encapsulated PCMs are often advised for pavement applications to overcome this problem. Encapsulation is wrapping or coating the PCM with an appropriate medium to prevent it from interacting directly with the paving materials [10]. Apart from expanded clay aggregates (ECA), which have been used in this work, silicon powder [11], polypropylene [12], expanded graphite [13], expanded perlite [14], Ceramisite [15], and acrylic outer shell [16] is the commonly explored encapsulating mediums for PCMs in pavement applications.
In the present study, the effect of PCM incorporation on the thermal and mechanical properties of concrete pavements has been experimentally investigated. Further, a total enthalpy-based heat transfer model is presented to predict the thermal performance of these PCM-incorporated cool concrete pavements under field conditions and validated with experimental data. Moreover, the significance of the thermal properties of the encapsulating agent and concrete slab on the cooling potential has also been investigated.
2. MATERIALS AND METHODS
2.1. Phase Change Material
In this study, a commercially available paraffin-based organic PCM with a melting point of 42 to 45°C, as shown in Fig. (1). This PCM (OM 42) was procured from PLUSS Technology Pvt. Ltd., India. Table 1 presents the physical and thermal properties of the PCM as provided by the manufacturer.
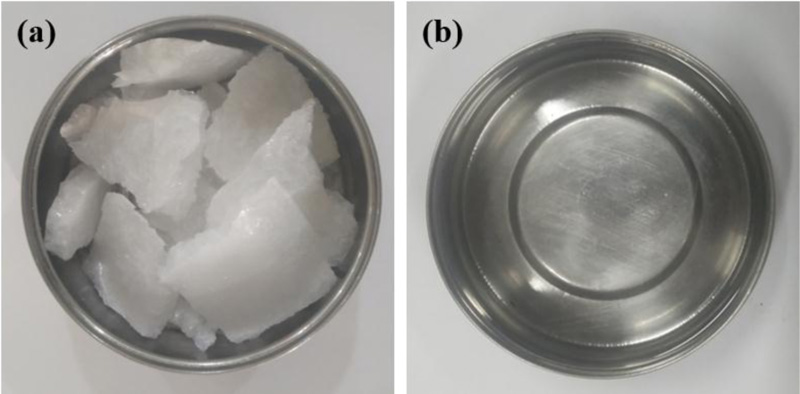
Material | Phase Change Temperature (˚C) | Liquid Density (kg/m3) | Solid Density (kg/m3) | Latent Heat (J/g) | Thermal Conductivity (W/mK) | |
---|---|---|---|---|---|---|
Melting | Freezing | |||||
OM 42 | 44 | 43 | 863 | 903 | 199 | 0.10 |
The PCM's phase change temperature and latent heat of fusion were measured using a differential scanning calorimeter (DSC) (Netzsch STA-449, Germany). The thermal stability of PCM-impregnated LWA was evaluated by thermogravimetry (TG) (Netzsch STA-449, Germany) under a nitrogen atmosphere at a heating rate of 10 °C per minute. The DSC curve of the PCM is shown in Fig. (2a). The PCM phase change initiates at 42°C with a peak of 53°C with a latent heat of 182.3 J/g. The TG result, shown in Fig. (2b), indicates that there is only negligible mass loss up to 200°C and 5% mass loss at 247.80 °C. Hence, OM 42 is thermally stable for pavement applications where the maximum temperature reaches 70 °C.
2.2. Expanded Clay Aggregates
ECA is a good PCM carrier material because it is highly porous and can absorb more than 40% of the liquid by its weight. The properties of ECA are presented in Table 2. ECAs with a mean diameter of 10 mm, (Fig. 3) were used in this study.
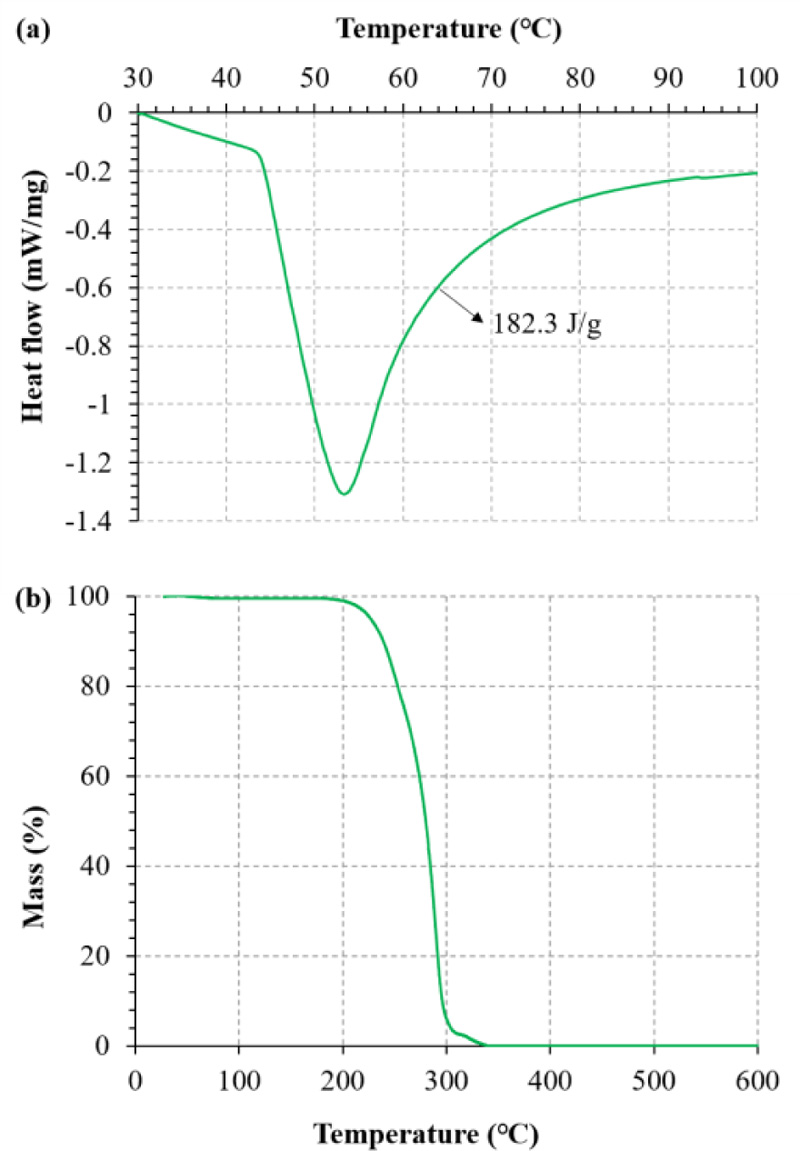
Mean Diameter | 10 mm |
---|---|
Dry density | 276.79 Kg/m3 |
Water absorption | 40.2% |
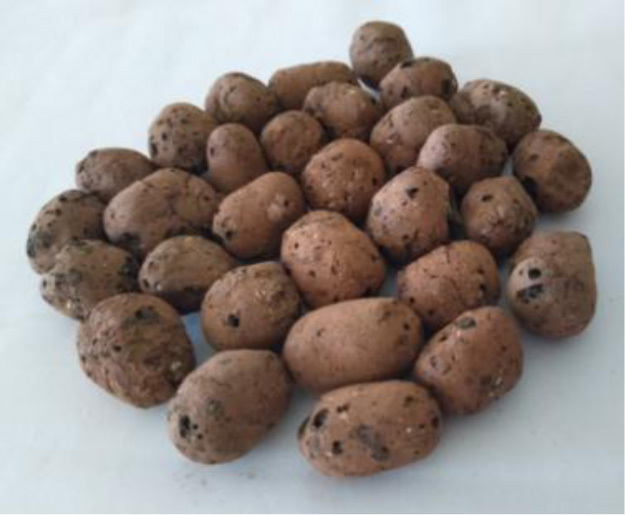
2.3. Coarse Aggregates
Locally available crushed stone aggregates with a maximum size of 20 mm and retained on a 4.75 mm sieve complying with the Bureau of Indian Standard (BIS) [17] were used as coarse aggregates in this study.
2.4. Fine Aggregates
River sand with a specific gravity of 2.66 and passing through IS 4.75 mm sieve complying with (BIS) [17] was employed as fine aggregates. The combined gradation curve of fine and coarse aggregates is shown in Fig. (4).
2.5. Cement
Ordinary Portland cement of grade 53, according to BIS [18], was employed in this work. The cement's initial and final setting times were 35 and 240 minutes, respectively.
3. METHODOLOGY
3.1. Experimental Methodology
3.1.1. Preparation of PCM Incorporated ECA
The ECAs were oven-dried for 24 hours to eliminate pore water before being impregnated with the PCM. For PCM abortion, the oven-dried ECAs were submerged in molten PCM for 24 hours. The ECAs were then filtered out of the molten PCM, and the surfaces were wiped with a towel. An ordinary Portland cement (OPC) coating was put over the ECAs to prevent PCM leakage. The PCM-impregnated ECA was immersed in cement paste and cured after coating to confirm effective coating. Fig. (5) shows a schematic illustration of this procedure.
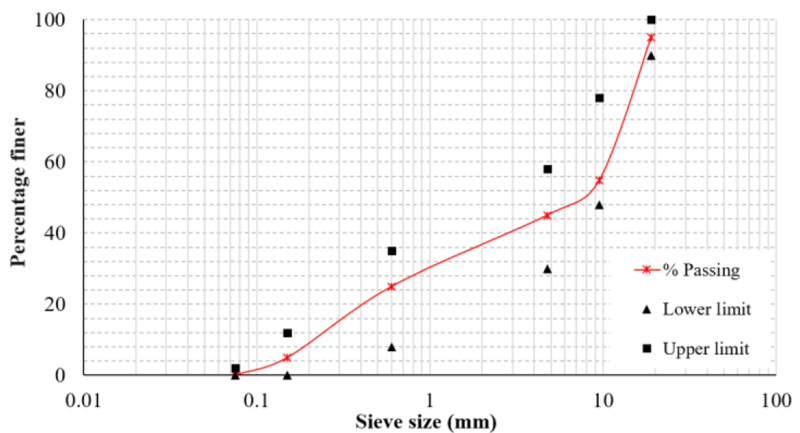
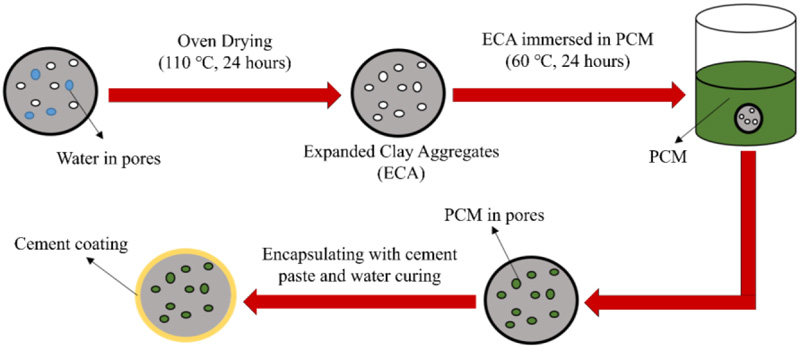
3.1.2. Mix Design
The concrete mix design was carried out according to Indian Roads Congress (IRC) specifications [19] to achieve a compressive design strength of 30 MPa. As per specifications, the M30 grade of concrete with a flexural strength greater than or equal to 3.8 MPa can be used for low-volume rural roads and urban roads with less truck traffic. The PCM-impregnated ECAs were incorporated as a partial replacement for coarse aggregates. The designed concrete exhibited desired mechanical strength with a replacement of coarse aggregates by the ECAs by up to 10% by weight. Hence, the mix with 10% coarse aggregate replacement was considered for the thermal analysis.
3.1.3. Thermal Performance Assessment
Two concrete slabs of 1m x 1m x 0.3m were cast using conventional and PCM-incorporated concrete to evaluate the thermal performance of PCM-integrated concrete pavements. Thermocouples implanted in the concrete and a data logger driven by solar energy were used to continuously monitor the pavement surface temperature under solar radiation. Because the top 100 mm of concrete pavement experience the highest temperature [20], the PCM-included concrete mix was only provided in the top 100 mm. A schematic representation of the temperature measuring setup is shown in Fig. (6).
3.2. Numerical Methodology
3.2.1. Physical Model
An in-house Finite Volume Method (FVM) based solver was developed for implementing the model. The numerical program was developed using FORTRAN. A 2-D rectangular domain of width 1 m and depth 0.3 m that contains PCM-impregnated ECA of diameter 0.01 m at the top 0.1 m was exposed to solar radiation (Q). The incoming solar radiation and thermal properties of the concrete, PCM, and ECA were the model's input parameters. The microvoids inside the porous ECAs are filled with PCM, called the impregnation of PCM in ECA. The top boundary is under the effect of conduction, convection, and radiation, and the sides were considered adiabatic Fig. (7). The pavement was assumed to resist over a 0.3 m sub base and the subgrade to define the lower boundary conditions. According to the studies of Gavin et al. [21], the ground temperature at 3.0 m below the surface is constant. Hence, the bottom boundary condition was defined as constant at a depth of 3.0 m.
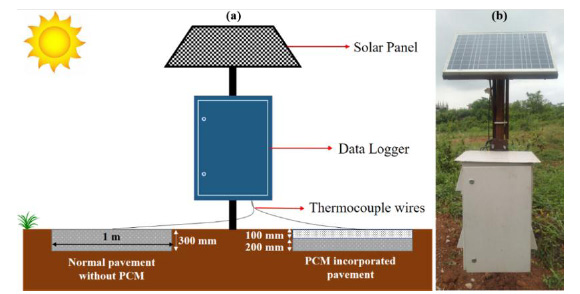
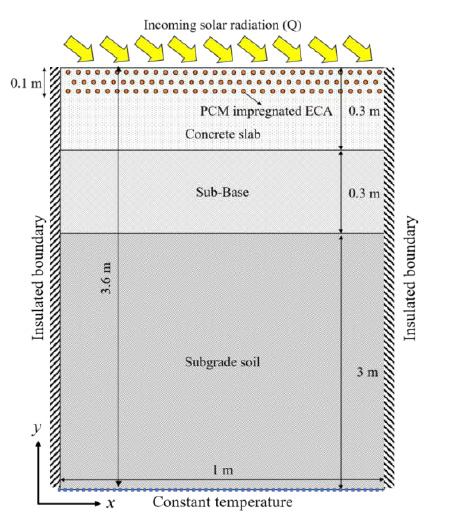
Under the dry condition, the pavement surface energy balance can be represented by Eq. 1.
![]() |
(1) |
As the wind speed has the same effect on the control slab (concrete slab with no PCM) and PCM incorporated slab, it was excluded from further calculations. Hence, Eq. 1 can be further expanded as,
![]() |
(2) |
Where Q is the incoming solar radiation, T is the temperature of the ground, Ts is the pavement surface temperature, Tsky is the sky temperature, k is the thermal conductivity of the pavement layers, ε is the emissivity of the pavement surface, and σ is the Stefan-Boltzmann constant.
The heat transfer between the pavement surface and underlying layers was considered conductive with no thermal contact resistance. The net heat energy stored in the domain is the summation of sensible and latent heat stored. The sunlight is directly incident on the top of the pavement surface, and the temperature gradient between the top and bottom surface initiates a heat flow through the domain from top to bottom. This increases sensible heat energy stored, followed by phase change of the PCM and latent heat storage. The thermophysical properties of concrete, subbase, subgrade, and ECA are given in Table 3.
3.2.2. Heat Transfer Model
Two different heat transfer models were used here to obtain the thermal field in the whole domain. A conduction heat transfer model was used to obtain the thermal field from concrete and sub-base domains. Conduction and diffusion-dominated phase change model is used to obtain thermal field from ECA-PCM regions. The phase change of microencapsulated PCM occurs inside the porous ECA, placed in the top 100 mm of the concrete slab. The following assumptions were made while developing the heat transfer model of the encapsulated PCM in ECA (µPCM-ECA) incorporated concrete pavements presented in this work.
- The initial temperature of the µPCM-ECA and the concrete slab is the same.
- The thermos-physical properties such as density, thermal conductivity, and specific heat of PCM, ECA, and concrete remain constant over the given temperature range.
- The isothermal phase change of the PCM.
- Equal sizes of porous ECA filled with PCM (µPCM-ECA) are homogeneously distributed in the top 100 mm of the slab.
- The ECA and PCM are in local thermal equilibrium.
- Volumetric change in µPCM during a phase change is negligible.
- A diffusion-dominated phase change of PCM is assumed inside porous ECA.
- The concrete slab is solid with homogeneous and isotropic properties, and thermal conduction heat transfer exists.
A conduction heat transfer model was used to obtain the thermal field in the pavement layers consisting of concrete, sub-base, and subgrade by solving the energy transport equation as Eq. 3.
![]() |
(3) |
The popular enthalpy model [24] was used to model PCM's phase change inside porous ECA. A thermal field in the domain is obtained by solving the energy equation based on the total enthalpy, consisting of sensible and latent heat content, as given by Eq. 4.
![]() |
(4) |
where (ρH)m is the volume-averaged total enthalpy expressed as given below.
![]() |
(5) |
where, ƒl and Hƒ is the melt fraction and latent heat of PCM, respectively. φPCM is the ECA porosity, and φg is the grid porosity. The PCM's melt fraction is the liquid PCM's volume in a representative elementary control volume consisting of PCM-impregnated ECA (ECA-μPCM) and concrete. ρm, Cpm and Km are the mixture density, specific heat, and thermal conductivity of the PCM-impregnated ECA (ECA-μPCM) and concrete. The average properties are then calculated based on the grid porosity of the main grid system consisting of porous ECA and concrete using Eq. 6 and 7.
![]() |
(6) |
![]() |
(7) |
ρECA -µPCM, CpECA -µPCM and KECA -µPCM are the mixture density, specific heat, and thermal conductivity of the PCM-impregnated ECA (ECA-μPCM).
ρCRT, CpCRT and KCRT are the mixture density, specific heat, and thermal conductivity of concrete. It should be noted that the mixture volumetric heat capacity is calculated based on the linear interpolation technique, while the thermal conductivity is calculated based on the harmonic mean interpolation. The harmonic mean interpolation accurately captures the jump discontinuity of the thermal conductivity between two dissimilar materials. The volumetric average thermal capacity of PCM-impregnated porous ECA was calculated using the ECA porosity (φPCM) as given in Eq. 8.
![]() |
(8) |
The effective thermal conductivity of the PCM-impregnated ECA was calculated using the modified Maxwell relationship [25], as given by Eq. 9.
![]() |
(9) |
4. RESULTS AND DISCUSSION
4.1. Experimental Results
Variations in pavement surface temperatures were tracked continuously for a year. Fig. (8) shows a reduction in pavement surface temperature due to PCM inclusion during the one year. During this time period, the annual average values of the relative humidity, air temperature, wind speed, solar radiation, and rainfall were observed to be 60.93%, 27.48 °C, 2.36 m/s, 204.77 W/m2, and 0.21 mm, respectively. The largest drop in pavement surface temperature measured with PCM integration is 4.12 °C, as shown in Fig. (8b). Furthermore, PCM inclusion resulted in a 2.24 °C reduction in annual average pavement surface temperature, which is a considerable reduction. Hence it is evident that the PCM incorporation is an effective method to reduce the pavement surface temperature, and also, the selected PCM is suitable for concrete pavement application in the study area.
However, the increased temperature of PCM-incorporated pavements at night remained a constraint, which requires further investigation. The higher pavement temperature at night may contribute to nocturnal UHI, defined as a rise in urban temperature at night [26]. As a result, PCM-incorporated pavements are not ideal for areas where night-time temperatures remain high. Therefore, PCM-incorporated pavements may be coupled with unidirectional heat transfer pavements to address this issue [27, 28]. In this, the thermal conductivity of the bottom layer of such pavements is kept higher than that of the top layers. This thermal conductivity gradient ensures heat conduction from the top to the bottom of the pavement. Also, the lowest pavement layer (subgrade soil) has a higher moisture content, which acts as a heat sink, allowing heat energy stored in lower levels to be dispersed rather than discharged into the atmosphere [7]. This will be taken into account in future investigations.
4.2. Numerical Analysis
4.2.1. Model Validation
The reduction in pavement surface temperature for a particular day was simulated, and the results were compared with experimental data collected from the site for the same day to validate the model. Fig. (9) presents the validation of the numerical model validation using experimental data acquired from the site. It may be seen that the model predicts the temperature variation quite accurately.
4.2.2. Effect of Thermal Conductivity of Concrete
Lower thermal conductivity of concrete may hinder the heat transfer and effective melting of PCM. The effect of the thermal conductivity of concrete on the cooling potential was analyzed, and the results are shown in Fig. (10). An increase in the cooling potential of PCM-incorporated concrete pavements was observed with an increase in the thermal conductivity of concrete slab (Fig. 10). As the thermal conductivity of concrete increased from 1.2 to 2.4 w/mK, the maximum reduction in pavement surface temperature increased from 4.40 to 7.12 °C. The effective melting of PCM and increased heat transfer to the lower pavement layers due to higher thermal conductivity may be the reason behind this trend. Adding thermally conductive additives such as steel fibers is a potential option to enhance the cooling potential. Hence, the study suggests the incorporation of thermally conductive additives along with PCM to enhance the cooling potential of concrete pavements.
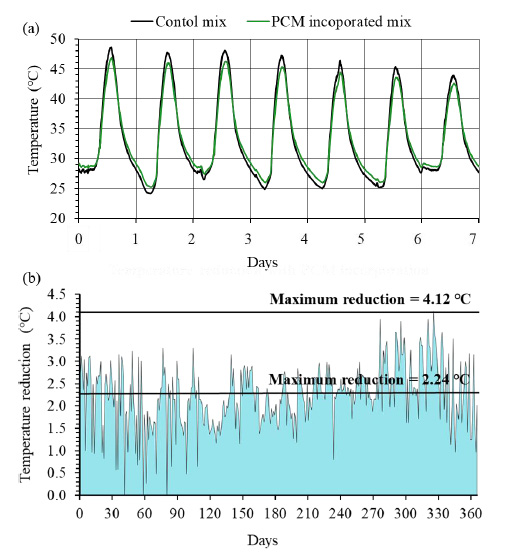
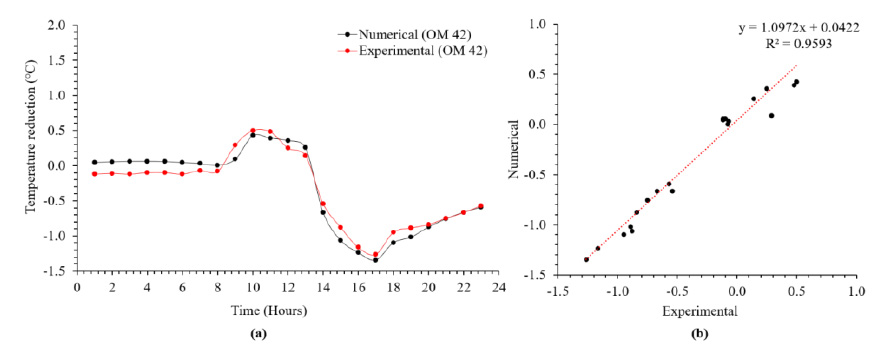
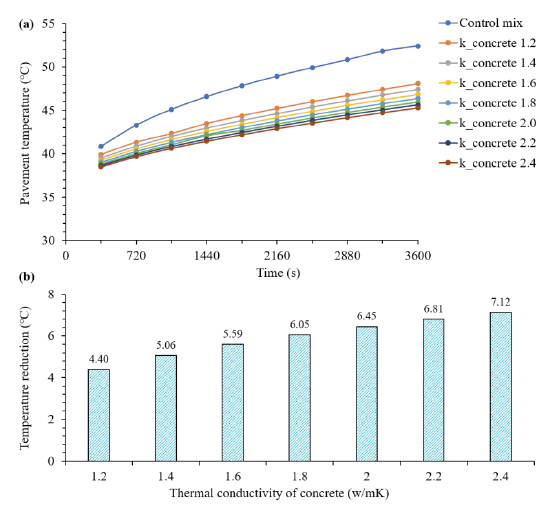
4.2.3. Effect of Porosity of Encapsulating Material
A reduction in pavement surface temperature was observed with an increase in ECA porosity. Fig. (11) shows the change in pavement surface temperatures with an increase in the porosity of the encapsulating material (ECA). While the addition of ECA of porosity 0.1 resulted in a temperature reduction of 1.04 °C, the addition of ECA having a porosity of 0.9 resulted in a temperature reduction of 5.68 °C. This improved thermal performance of the pavement slab is attributable to the increased PCM content held in the encapsulating medium due to the higher porosity. Achieving a porosity of 0.9 is challenging. However, retaining the encapsulating medium's porosity as high as feasible is advantageous. Depending on the cost constraints and the desired temperature reduction, core-shell encapsulation can also be used.
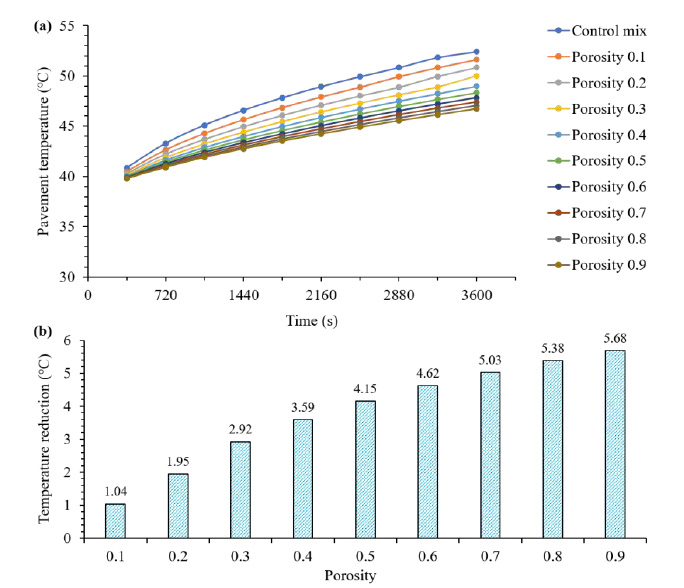
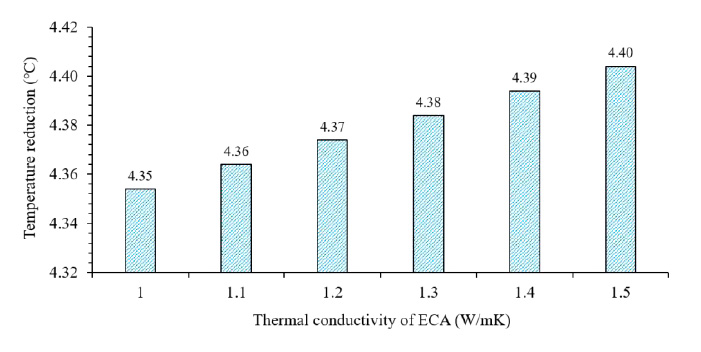
4.2.4. Effect of Thermal Conductivity of Encapsulating Material
Increasing the encapsulating material's thermal conductivity would result in the effective melting of the PCM and an increase in the pavement cooling potential. However, the findings Fig. (12) show that the thermal conductivity of the encapsulating medium improves the cooling potential of PCM-integrated pavement slabs, but the effect is minimal.
CONCLUSION AND RECOMMENDATIONS
The impact of incorporating PCM on the pavement surface temperature was studied experimentally. A numerical model was also developed and verified using experimental findings to assess the thermal performance of PCM-integrated concrete pavements. The following are the key findings of this study:
- PCM incorporation in concrete pavements is an effective method to achieve cool pavements. The PCM (OM 42) incorporation resulted in a 2.24 °C annual average temperature reduction, with a maximum of 4.12 °C.
- The PCM leakage is significantly reduced when cement paste is applied to the ECA; therefore, it is possible to overcome the negative impacts of PCM on concrete hardening.
- With a higher thermal conductivity of the concrete slab, effective melting of PCM and better heat transfer to the lower pavement layers can be achieved. The maximum drop in pavement surface temperature increased from 4.40 to 7.12°C when the thermal conductivity of concrete increased from 1.2 to 2.4 W/mK. Increased concrete thermal conductivity leads to increased PCM melting and efficient heat transfer to the lower pavement layers, increasing cooling potential.
- More PCM may be accommodated with increased porosity of the encapsulating medium, leading to improved cooling performance. With the addition of ECA of porosity 0.1, the pavement surface temperature can be reduced by 1.04 °C, whereas with porosity of 0.9, the temperature can be reduced by 5.68 °C.
- An increase in the thermal conductivity of the encapsulating medium will result in effective melting and enhanced cooling performance. However, the improvement in cooling performance is negligible.
Conventional pavements may often be preferred over PCM-incorporated pavements due to their low initial costs and lower construction time. However, this market barrier can be overcome by improving the efficiency of PCM-incorporated pavements. Based on the findings, the research proposes increasing the porosity of the encapsulating medium and thermal conductivity of PCM incorporated concrete slab for better performance, considering the target reduction and cost constraints. If necessary, core-shell encapsulation can be used. As the thermal characteristics of the encapsulating material have a minor impact on cooling potential, they may be neglected.
LIST OF ABBREVIATIONS
ECA | = Encapsulating Material |
Q | = Solar Radiation |
FVM | = Finite Volume Method |
IRC | = Indian Roads Congress |
CONSENT FOR PUBLICATION
Not Applicable.
AVAILABILITY OF DATA AND MATERIALS
The data supporting this study's findings are available from the corresponding author [B.R.A] on request.
FUNDING
None.
CONFLICT OF INTEREST
The authors declare no conflict of interest, financial or otherwise.
ACKNOWLEDGEMENTS
Declared none.